- Quantum Information and Quantum Technology
- Quantum Gravity and Gauge/Gravity Correspondence
- Gravitational Waves and Cosmology
- Quantum Matter
- Strongly Coupled Quantum Systems and (Quantum) Computing
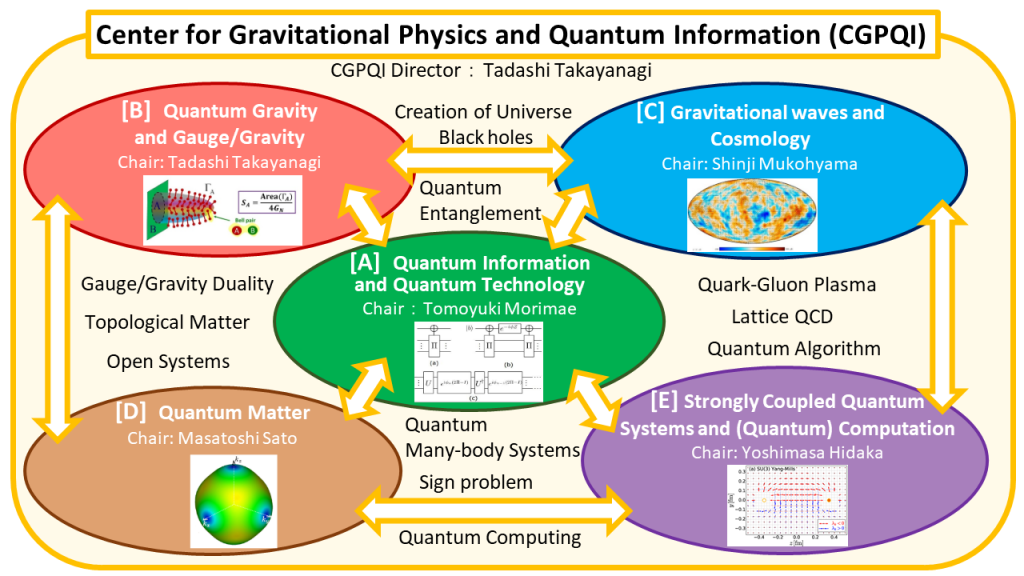
Quantum Information and Quantum Technology
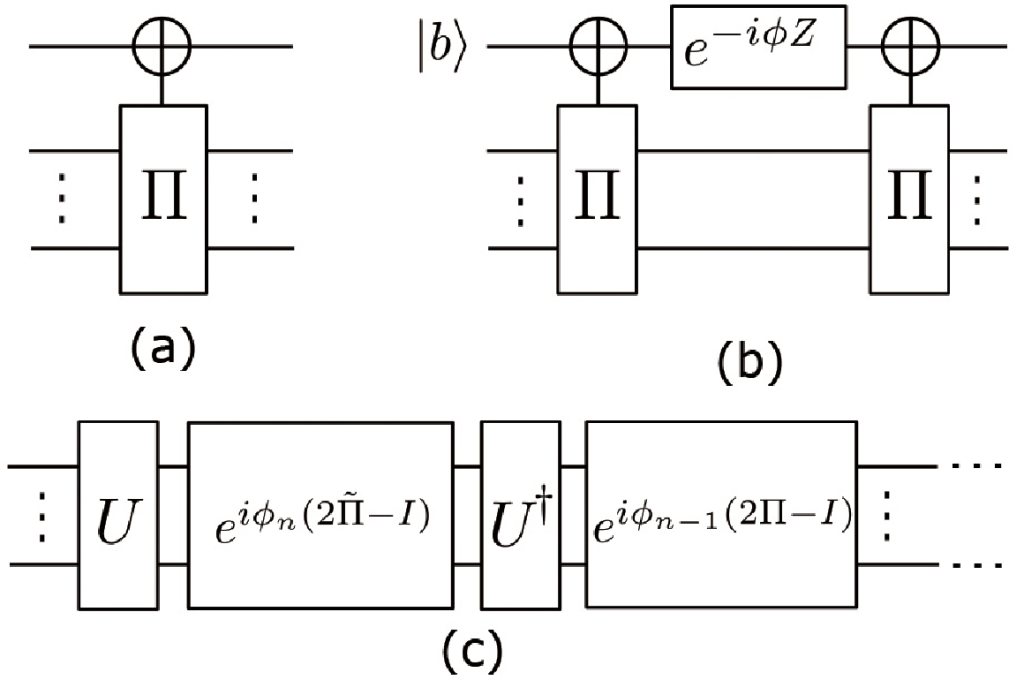
The microscopic world of atoms, molecules, and light is described by a physical theory called quantum theory. This quantum theory is known for the appearance of many phenomena, such as quantum superposition and entanglement, that are very strange from the perspective of the macroscopic world in which we normally live, and understanding their mysterious nature has been an important issue in physics since ancient times.
Quantum information is the study that aims to realize unprecedentedly high performance information processing technology by successfully controlling the mysterious nature of quantum theory. In fact, it is now known that it is possible to realize ultra-high-performance computers (quantum computers) that far surpass the computing power of current computers (classical computers), as well as cryptographic tasks with new functions that cannot be realized with various types of classical computers, and many theoretical and experimental studies have been conducted worldwide. One of the goals of this division is to promote the study of this quantum information. In particular, we will work on topics such as quantum computational complexity theory, quantum algorithm theory, quantum cryptography, and quantum error correction theory.
On the other hand, another important goal of quantum information is to approach a deeper understanding of theoretical physics from a completely new perspective by feeding back new results, concepts, techniques, etc. obtained through research on quantum information to traditional physics. In fact, the results of quantum information have recently been imported back into statistical physics, condensed matter, elementary particles, gravity, and other fields, and researchers in these fields and quantum information are actively exchanging information. Conversely, there are also cases in which something that has been studied for many years in physics is useful in quantum information. For example, tensor networks, which have long been studied for efficient numerical simulation of quantum many-body systems, are also useful for classical simulation of quantum computation (i.e., to see how much better quantum computation is than classical computation). Thus, in this new area where physics and information science intersect, research in this research division is being conducted in both directions, from physics to information and from information to physics.
Quantum Gravity and Gauge/Gravity Correspondence
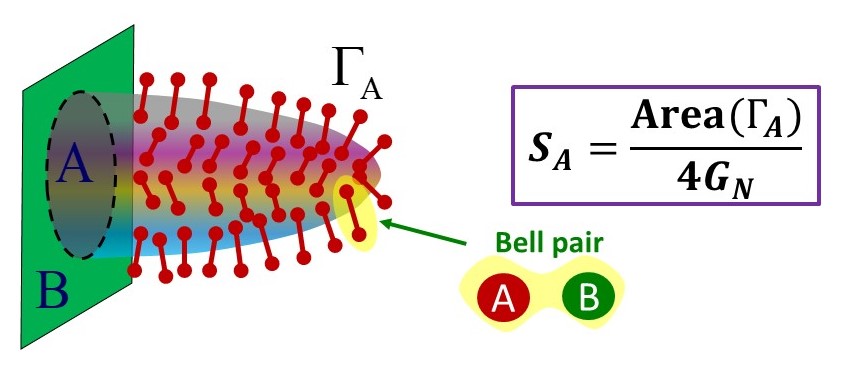
There are four types of forces acting in nature: electromagnetism, weak interaction, strong interaction, and gravity. Of these, the electromagnetic force, weak interaction, and strong interaction are understood in a unified manner using the Standard Model in quantum theory, which describes fundamental laws in the microscopic world. However, with regard to gravity, although the description by general relativity has been established as a law in the macroscopic world, the quantum theory of gravity (quantum gravity) is still one of the most difficult problems in physics, as its laws are still unresolved. Superstring theory is a theory obtained by considering the smallest unit of all things to be a string, rather than a particle, and is well known as a promising candidate for quantum gravity.
From the research on superstring theory, a new idea of "Gauge/Gravity duality” emerges. This is a phenomenon in which "a theory of gravity becomes equivalent to a theory of quantum matter (such as a gauge theory) that does not include any gravitational interaction”. By using this, it is expected that the difficult theory of quantum gravity may be formulated in a non-perturbative manner as a more familiar theory of the quantum many-body system. Furthermore, it has become clear that quantum information theory plays an important role behind this mysterious phenomenon. It has been found that the strength of quantum entanglement( so called entanglement entropy), which represents the correlation of quantum information in quantum many-body systems, is proportional to the cross-sectional area of the universe of gravity theories, via the Gauge/Gravity duality. For example, we can understand the Einstein equation, which governs the fundamental dynamics of gravity, as a thermodynamic property of entanglement entropy. By analyzing the Gauge/Gravity duality using this method, we can also obtain the prediction that the structure of quantum entanglement in theories of quantum matter (gauge theories) corresponds to the geometry of the gravitational universe. One promising direction which realizes this idea is the conjecture that the spacetimes in gravity may be regarded as the networks of quantum entanglement which appear in the tensor networks which describe quantum states in many-body systems.
An important target that is expected to be solved by quantum gravity, is the black hole information problem. Black holes are thought to gradually shrink in size and eventually evaporate due to thermal radiation (Hawking radiation). What happens to the information hidden inside the black hole at that time? This problem has remained intractable for a long time, but only recently has it become one of the central themes internationally, as it was found that a fusion of quantum information theory and Gauge/Gravity duality can provide a clue to this problem. This is one of the main topics studied in this group.
Also, the ultimate goal of quantum gravity is to answer the question, how did the universe we live, was created ? Though the dynamics of time-dependent quantum gravity theory is important in this context, the current understanding of superstring theory is still far from elucidating this issue. The aforementioned idea of Gauge-Gravity correspondence is applicable to the anti de-Sitter universe, which has a negative cosmological constant, and cannot deal with the real universe in which the cosmological constant is considered to be positive. However, if the basic principle of Gauge-Gravity correspondence can be understood using quantum information theory as a key, it is expected to be greatly generalized so that it can be applicable to the universe with a positive cosmological constant.
To understand quantum gravity, discrete theoretic approaches are also useful, which provides complementary viewpoints. It would be a common naive fundamental question, “Is it possible to describe spacetime and universe by some fundamental discrete elements, similarly as the atoms formulating matters ?” It is a very non-trivial question whether a discrete theory would be able to formulate quantum gravity. In particular, it is critical whether the continuous spacetime in general relativity could be derived as an infrared effective description of a discrete theory. This is nothing but studying the birth of universe in a discrete theory. Moreover, it would also be a stimulating possibility that the black hole entropy could be counted by discrete degrees of freedom. In fact there exist various discrete approaches to quantum gravity, and one approach studied in this center is the tenor model, which is a higher dimensional extension of the matrix model, that is successful in describing two dimensional quantum gravity.
The purpose of this research group is to bring a new development in quantum gravity by fusing above diverse approaches in theoretical physics with the methods of quantum information theory, and to become a central axis in the research of quantum gravity in Japan.
Gravitational Waves and Cosmology
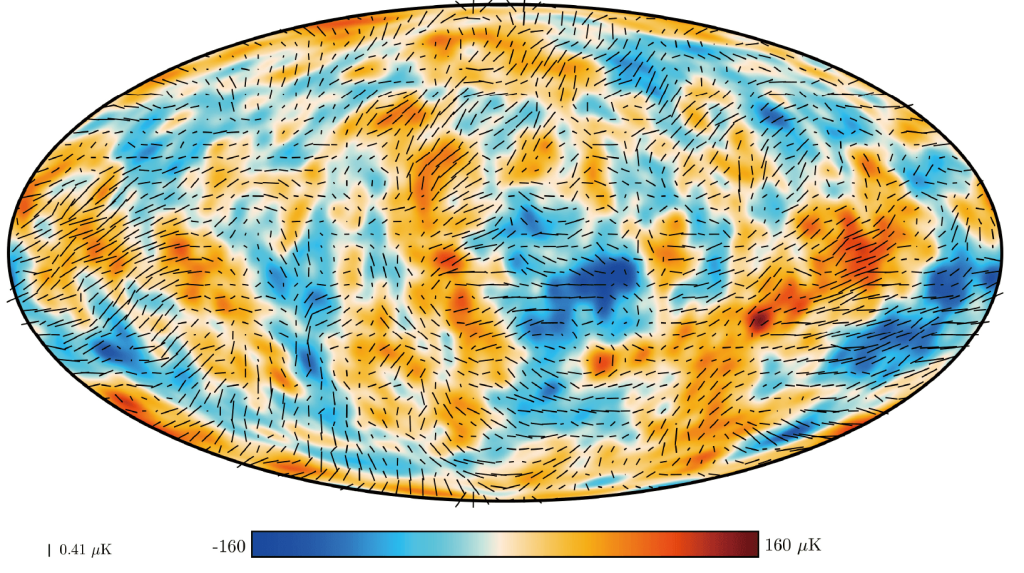
Cosmology has been rapidly developing, based on precision observational data. It is fair to say that many parameters describing our universe have been determined, or at least are in the process of being determined, with good precision. However, the physics behind the values of these parameters is still hidden in a veil of mystery. For example, we do not know what dark energy and dark matter really are, although our universe is thought to be filled mostly with them. Also, what made our universe so big? This question can be addressed by cosmic inflation, but again we do not know the physical origin of the inflaton field driving inflation. Three great mysteries, dark energy, dark matter and inflation, are standing in the way of cosmology which boasts precision observational data. We need to continue tackling the mysteries of the universe by using every possible means such as general relativity, statistical physics, particle physics and superstring theory.
In our universe physical phenomena at various scales occur constantly, having mutual influence on each other. We consider it necessary for physics at the largest scales, i.e. cosmology, to be connected with physics at the shortest scales. This is because the extremely high energy state of the early universe makes microscopic physics essentially important. At the beginning of the universe, both gravity and quantum effects are significant and, as a result, both general relativity and the conventional quantum field theory break down. For this and many other reasons, we need a theory of quantum gravity that can describe gravity in a quantum mechanically consistent way. Cosmology based on quantum gravity should play important roles in our understanding of the universe.
The cosmological constant (cc) problem is one of the most difficult problems in theoretical physics and cosmology. There are two aspects of the cc problem. The first is "Why small?" The observational upper bound on the cc is smaller than what is naively expected quantum mechanically by 120 orders of magnitude. At present there is no theory that can convincingly explain this small value. For this reason, some researchers show a tendency to rely on the anthropic principle. However, we consider it premature to give up seeking a solution without the anthropic principle. If a new theory that solves the cc problem based on physical principles is found then the anthropic "solution" will soon be forgotten. We should not give up attempting difficult problems.
The second aspect of the cc problem is "Why not zero?" and "Why now?" This is nothing but one of the three great mysteries of the universe, dark energy. Although dark energy is thought to fill more than 70% of the present universe, we do not know what it really is. This situation makes us feel that a hint for new physics may be hidden in gravity at cosmological scales. Evidences for dark energy so far are based on indirect observations of gravity at long distance and time scales but gravity at such scales has never been measured directly. When the perihelion shift of Mercury was discovered in the Nineteen Century, some people tried to explain this interesting phenomenon by introducing an unknown planet, so to speak dark planet. Some people even "discovered" it. As we all know, however, the right answer was not a dark planet but to change gravity, from Newton's theory to Einstein's general relativity. This was indeed the beginning of the success of the new theory. With this historical fact in mind, it is understandable that many researchers now think that the mystery of the accelerated expansion of the universe might be similar. People thus recently started to ask "Can we change general relativity at long distance or/and time scales instead of introducing dark energy or/and dark matter?" Needless to say, however, it is observations and experiments that give a decision. To answer the question "dark energy or modification of gravity?", we need to construct a theoretically consistent and observationally viable theory of gravity and derive verifiable predictions.
In September, 2015, US gravitational wave detectors, advanced LIGO, achieved the first direct detection of gravitational waves emitted from binary black holes. Japanese gravitational-wave community has developed another gravitational wave detector, named KAGRA, and it started the observational run in 2020. As a result, we will be able to learn in depth the strong self-gravitating phenomena, which are still poorly known. In addition, gravitational-wave observation will give a strong impact on the high-energy astrophysics. For example, if a core collapse supernova explosion or a gamma-ray burst occurs in the nearby galaxies, gravitational waves will be observed and we will get rich information for these phenomena, which are also poorly understood. Furthermore, the gravitational-wave observation will be used for testing general relativity. If we are lucky, we may be able to get evidence for the violation of general relativistic prediction in detected gravitational waves.
In the gravitational-wave astronomy, the role of theoretical researchers is quite important. The reason for this is that for the detection of gravitational waves from noisy data and for the determination of the parameters of gravitational-wave sources, we need a highly accurate gravitational wave templates by analyzing Einstein's equation. Developing efficient data-analysis methods is also the urgent issues in this field.
It is also quite important to investigate electromagnetic signals that could be emitted together with the gravitational-wave signals. Indeed, the binary neutron star merger observation in 2017, the first gravitational and electromagnetic wave multimessenger detection, has allowed us to set stringent bounds on the speed difference between gravitational and electromagnetic waves and constraints on modified gravity theories. Coincident detection of electromagnetic signals with gravitational waves will also enhance the reliability of the gravitational-wave detection. Furthermore, electromagnetic signals will bring rich information which cannot be obtained only from gravitational wave signals. To establish the gravitational-wave astronomy, we have to also develop an efficient method for the detection of electromagnetic signals. For this task, theoretical prediction of electromagnetic signals is quite important.
The gravitational-wave astronomy has just begun and now is the exciting era. There are many topics to which theoretical physicist can contribute. One of the important roles of Center for Gravitational Physics and Quantum Information is to enhance the Japanese activity in the gravitational-wave astronomy as the theoretical physics center in Japan. The other important role is to encourage the international collaboration among a wide variety of researchers in the gravitational-wave astronomy.
Quantum Matter
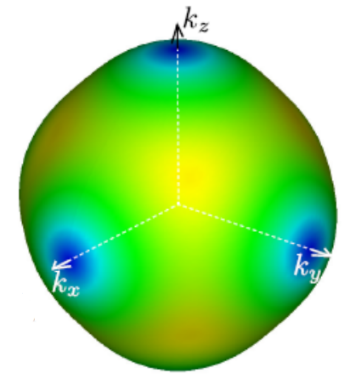
In short, the ultimate goal of condensed-matter theory to understand a variety of complex behaviors of matter with a combination of relatively simple models and fundamental principles of physics, and to predict new phenomena on the basis of the knowledge gained there. There are numerous examples of great success, e.g., the band-theoretic understanding of metals and insulators, microscopic theories of superconductivity and superfluidity, magnetism in metals, and they not only have been producing basic and ubiquitous concepts in physics but also underlie modern technologies that are indispensable to our daily life. Among those great advances in recent years is the understanding of the so-called ``topological materials’’, that has been initiated in 1980s in the study of quantum Hall effects and was the subject of the Nobel Prize in Physics in 2016. Prior to this, physics had already used topological idea (homotopy) successfully in classifying excitations and defects in (classical) ordered materials. However, in understanding the properties of topological materials, we focus on non-trivial topological properties of ``quantum-mechanical wave functions’’. In particular, since the discovery of the topological insulators and superconductors, it has been widely recognized that symmetries, e.g., time-reversal and particle-hole, play crucial roles in topological states of matter, and this dramatically deepened our understanding. Moreover, the research area of topological states of matter keeps expanding to include even systems that are apparently unrelated to quantum mechanics, e.g., mechanical systems, electrical circuits, and equatorial waves on the earth, and those described by non-Hermitian Hamiltonians.
However, being topological, those topological states of matter had been defying the characterization with ``local order parameters’’, that had been quite successful for conventional states, and this hampered systematic and essential understanding of them. The concept of ``quantum entanglement’’ born in the field of quantum information paved a new way; it tells us that there are two major categories of topological phases, which enable us to systematically distinguish between ``genuine topological phases’’ such as fractional quantum Hall phases and ``symmetry-protected topological phases’’ like topological band insulators. Since then, topological states of matter have been providing an active arena for interdisciplinary exchange between condensed-matter theory and quantum-information theory.
Quantum spin liquids, which have been theoretically proposed and actively studied around the end of 1980s in the context of high-temperature superconductivity, are (quantum) disordered magnetic states at zero temperature. Mainly because of the lack of reliable microscopic theories, quantum spin liquids have been the Holy Grail in quantum magnetism for a long time. However, since the beginning of the 21st century, our understanding of the spin liquids has become even deeper though the study of several solvable toy models, and now comprehensive study of spin liquids including the experimental verification of their non-trivial properties such as the quantized transport coefficients and excitations obeying (non-Abelian) fractional statistics is one of the big trends in condensed-matter physics.
Fruitful exchange between condensed-matter physics and quantum information is not restricted to the area of topological states of matter. Although numerical approaches are indispensable in strongly correlated quantum many-body systems in which the problem can hardly be treated exactly, all the methods have their own drawbacks or limitations, and a real breakthrough from a new perspective had been longed for in order to attack hard problems in modern condensed-matter physics. Again, through the study of the properties of entanglement that quantum many-body systems exhibit at low energies, an idea utilizing a new type of quantum wave functions (e.g., matrix-product states and tensor networks) that efficiently simulate the true many-body ground states has been proposed. Approaches based on this idea have been successfully applied to a variety of problems which had been extremely hard otherwise. Also, intimate connections between tensor network states and AdS/CFT has yielded a very active interdisciplinary study bridging among condensed-matter theory, quantum gravity, and quantum information.
Another major goal of condensed-matter/statistical physics that appears to be totally different from what was described above is to reveal universal laws which do not depend on particular systems or materials. Although statistical mechanics/thermodynamics that we learn at the undergraduate level is one of the successful examples, being limited to the vicinity of thermal equilibrium, their range of applicability is not at all wide. On the other hand, as Schrödinger pointed out in his book "What is life?", open non-equilibrium systems such as living organisms often decrease the entropy of the system of interest by consuming entropy into the environment. Since Schrödinger's proposal of negentropy, statistical thermodynamics of open non-equilibrium systems has been of great interest, but its general theory has not been established so far.
The most controlled open non-equilibrium systems are those in which extremely small systems, such as quantum dots, are connected to their environments. Although the effective Hamiltonian of these systems is non-Hermitian, there has been a growing interest in studying the thermodynamic properties of these small systems in more detail by directly addressing the quantum master equation. This approach seems to contradict the classical picture that thermodynamics is only valid in the limit of infinite degrees of freedom, but it can be assumed that the small system and its connected environments obey ordinary thermodynamic laws. On the other hand, when the system is connected to multiple environments, the temperature and chemical potential of each can be different, and a non-equilibrium steady state with heat flow and electric currents flowing through the system is easily realized. Again, entropy plays a major role, and it is possible to discuss, for example, a heat engine that controls the parameters of a small system or environments, and there is hot debate about whether entanglement and a quantum effect can increase thermal efficiency or power. Also, in a heat engine, the control parameters are cyclically modulated in parameter space, so if there are multiple control parameters, their geometrical properties create exotic effects that directly affect the performance of the heat engine. Quantum thermodynamics of such open systems can be one hot topic of the Quantum Gravity Center.
Traditionally, statistical thermodynamics of open systems has been concerned with classical systems rather than quantum systems. This is because classical systems have the advantage that they are relatively easy to control and their results are easily visible. Also, many of the targets of biophysics, which Schrödinger opened the door to, are macroscopic systems that exist in high-temperature environments that can be treated classically. How matter flows, solidifies, and characterizes itself in these classical nonequilibrium systems has been discussed independently of quantum gravity, but quite recently some attempts been made to explain yield transitions in classical particle many-body systems such as granular materials from the holographic principle, and the trend and can no longer be unrelated to it. In addition, in both quantum and classical open systems, relative entropy (Kullback-Leibler divergence), Fisher information content, etc. are key to understanding and controlling these systems, and information theoretic treatment has become indispensable. The nature of this general method is not dependent on the individuality of the material, and it can provide a common modern viewpoint regardless of the field of expertise. By expanding such a world view, the field of statistical physics/condensed matter physics can contribute to the development of Quantum Gravity Center.
Strongly Coupled Quantum Systems and (Quantum) Computing
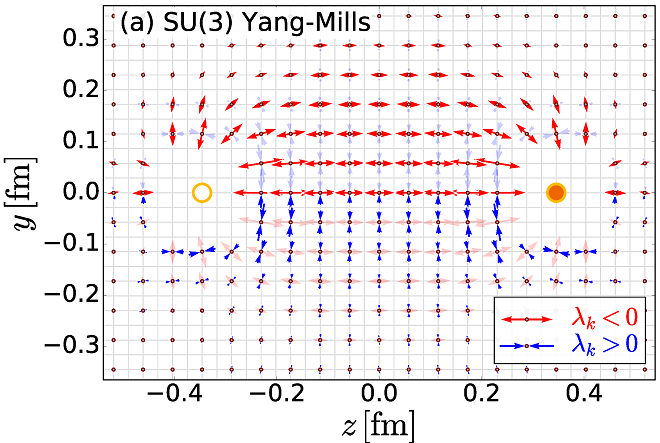
Matter composed of quarks and gluons (quark-gluon plasma, QGP) underwent the last vacuum phase transition in the early universe, creating the present universe composed of protons, neutrons, and electrons. Around the phase transition temperature, QGP is almost a perfect fluid, and can be said to be strongly coupled matter with a viscosity of η=(1-3)s/4π, which is close to the minimum shear viscosity (η=s/4π, where s is the entropy density) predicted by the superstring theory.
While the most famous example of a strongly coupled system is the strongly correlated electron system, as in the above example, quark-gluon matter described by quantum chromodynamics (QCD), as well as hadrons and nuclei composed of quarks and gluons, are also strongly coupled systems. For example, the QCD vacuum is not an "empty" state with no particles, but consists of many particle pairs induced by interactions. Gluons take various topological configurations such as instantons and monopoles to cause color confinement. Quark-antiquark pair production and annihilation continuously take place and chiral symmetry is spontaneously broken, which makes the constituent quark masses finite. The mass thus obtained is about 100 times greater than the mass produced by the Higgs boson, so that more than 99% of the visible matter that makes up our world is created by the condensation that results from interactions.
In order to describe such strongly coupled systems, a non-perturbative theoretical framework is necessary. In the case of the QCD vacuum described above, the lattice QCD simulations, in which quarks and gluons are placed on a lattice with finite degrees of freedom, are used to perform rigorous calculations. Lattice QCD has met great successes to obtain hadron masses, various transition matrix elements, transition temperatures to QGP, and equations of state for high-temperature matter. Some of these results explain experimental data beautifully. The transition temperature and equations of state are not direct observables from experiment, but have been utilized in fluid dynamics and used to describe high-energy heavy-ion collisions. Furthermore, progress has been made in predicting hadron-hadron interactions, which were not known before but examined in recent experiments. While these are issues in classical computing, they are important for the progress in physics and are among the issues to be addressed at the Center.
However, the Monte Carlo method, which has been mainly used in numerical simulations of lattice QCD, is not a panacea, and in situations where there is a problem called the sign problem, the computational complexity increases explosively, and in principle, efficient simulations are not possible. It is known that the sign problem often appears in the presence of topological terms and chemical potentials or in the real-time evolution of quantum systems. They involve very interesting physics, such as the phase structure of finite density QCD matter inside neutron stars, and quantum dynamics in the early universe. Therefore, it is an important challenge in modern physics to develop new computational methods that are effective even in the presence of sign problems.
Recently, quantum computer-based approaches have been attracting attention as a way to overcome this situation. While in ordinary lattice QCD simulations, numerical calculations of path integrals are performed based on the Lagrangian formalism, this approach uses the Hamiltonian formalism. In the Hamiltonian formalism, the sign problem does not exist from the beginning because the physical quantities are not represented by path integrals. As a compensation, however, the numerical simulation requires the handling of very large vectors and matrices corresponding to states and operators respectively, which is considered to be very computationally time-consuming on ordinary classical computers. Therefore, it is expected that such problems may be solved in a short time by using future quantum computers. In reality, there are still various issues to be solved, such as the fact that the resources of quantum computers have not yet been fully developed and that effective methods for analyzing QCD with quantum computers have not yet been established. We are conducting research to solve these problems.